NASA MEPAG Report: Mars Science Goals, Objectives, Investigations, and Priorities: 2006
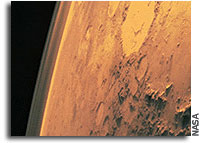
February 10, 2006
Prepared by the MEPAG (Mars Exploration Program Analysis Group) Goals Committee:
John Grant, Chair, Smithsonian Institution (grantj@si.edu)
Representing Goal I
Jan Amend, Washington University (amend@levee.wustl.edu)
Andrew Steele, Carnegie Institute of Washington (steelie@mac.com)
Representing Goal II
Mark Richardson, Caltech (mir@gps.caltech.edu)
Steve Bougher, University of Michigan (Bougher@engin.umich.edu)
Representing Goal III
Bruce Banerdt, Jet Propulsion Laboratory (bruce.banerdt@jpl.nasa.gov)
Lars Borg, University of New Mexico (lborg@unm.edu)
Representing Goal IV
John Gruener, Johnson Space Center (john.e.gruener@nasa.gov)
Jennifer Heldmann, Ames Research Center (jheldmann@mail.arc.nasa,gov)
Comments or concerns regarding this document should be addressed to Dr. John Grant, Chair, MEPAG Goals Committee (grantj@si.edu).
This report has been approved for public release by JPL Document Review Services (CL#05-2215) and may be freely circulated.
Recommended bibliographic citation:
MEPAG (2006), Mars Scientific Goals, Objectives, Investigations, and Priorities: 2006, J. Grant, ed., 31 p. white paper posted February, 2006 by the Mars Exploration Program Analysis Group (MEPAG) at http://mepag.jpl.nasa.gov/reports/index.html.
PREAMBLE
In 2000, the Mars Exploration Program Analysis Group (MEPAG) was asked by NASA to work with the science community to establish consensus priorities for the future scientific exploration of Mars. Those discussions and analyses resulted in a report entitled Scientific Goals, Objectives, Investigations, and Priorities, which is informally referred to as the “Goals Document” (MEPAG 2001 1). The initial report proved to be very useful for guiding program implementation decisions. It also has become clear over the past few years that the report requires regular updating in light of dramatic new results from Mars and evolving high-level strategic direction from NASA. For this reason, MEPAG periodically revises the Goals Document (MEPAG, 20042; MEPAG, 20053; MEPAG 2006-this document). As was the case with previous versions, the Goals Document is presented as a statement of community consensus positions.
The MEPAG Goals Document is organized into a four-tiered hierarchy: goals, objectives, investigations, and measurements. The goals have a very long-range character and are organized around major sectors of scientific knowledge. Specific statements of these goals are found in this report, but they are commonly referred to as Life, Climate, Geology, and Preparation (for Human Exploration). Because developing an understanding of Mars as a system requires making progress toward meeting all four goals, MEPAG has not attempted to prioritize the goals, but rather represents them equally.
The four goals each include 2-3 objectives that embody the strategies and milestones needed to achieve them. Objectives are presented in priority order, because there is often an order in which the scientific questions can most logically be resolved, and/or some objectives are perceived to be more important than others. In the present version of the Goals Document, there are a total of 10 objectives, eight of which are scientific in nature, and two of which relate to safe mission operations.
A series of investigations that collectively would achieve each objective is also identified. The investigation structure is specifically intended to apply to the scientific objectives, but a breakdown is also presented for the two engineering objectives. While some investigations can be achieved with a single instrument, others will require multiple instruments on multiple missions. Each set of investigations is independently prioritized for each objective.
1 MEPAG (2001), Scientific Goals, Objectives, Investigations, and Priorities, in Science Planning for Exploring Mars, JPL Publication 01-7, p. 9-38. Available on the web at http://mepag.jpl.nasa.gov/reports/index.html.
2 MEPAG (2004), Scientific Goals, Objectives, Investigations, and Priorities: 2003, G. J. Taylor, ed., 23 p. white paper posted 07-16-04 by the Mars Exploration Program Analysis Group (MEPAG) at http://mepag.jpl.nasa.gov/reports/index.html.
3 MEPAG (2005), Mars Scientific Goals, Objectives, Investigations, and Priorities: 2005, 31 p. white paper posted August, 2005 by the Mars Exploration Program Analysis Group (MEPAG) at http://mepag.jpl.nasa.gov/reports/index.html.
Measurements constitute the fourth tier of the MEPAG hierarchy. Measurements are made by instruments, which are tangible objects that can be built and flown to Mars. MEPAG has only considered scientific objectives that can be traced down to measurements (i.e., theoretical modeling was not considered). The Goals Document is presented as a statement of community consensus positions and it is MEPAG’s intent that the descriptions of scientific objectives and investigations serve simply as example targets for future instrument development and measurements. As measurement capabilities and techniques evolve, detailed requirements should to be defined by Principal Investigators, Science Definition Teams, and Payload Science Integration Groups for program missions and by the Principal Investigator and Science Teams for Scout missions. These requirements can then contribute to forward program planning. An important exception to this strategy, however, is the measurement set associated with Goal IV Objective A, which relates to environmental data sets necessary to reduce the risk of future human missions to acceptable levels. In that case, a clear criterion exists (degree of impact on risk reduction) that enables those measurements to be listed in priority order.
Completion of all the cited investigations will require decades of studying Mars and it is possible that many investigations may never be truly completed (even if they have a high priority). Thus, evaluations of prospective missions and instruments should be based on how well the investigations are addressed. While priorities should influence which investigations are conducted first, they should not necessarily be done serially, except where it is noted that one investigation should be completed first. In such cases, the investigation that should be done first was given a higher priority, even in where it is believed that a subsequent investigation will be more important.
Some types of Mars-related scientific research take place without flying spacecraft to Mars. Most notably, these include meteorite studies, telescopic observations, theoretical models, and fundamental research of diverse character. The Goals Document does not consider these sectors of research in its hierarchy, or in its prioritization system.
Some general thoughts on mission technology planning
Even a cursory reading of the goals, objectives, and investigations will reveal several crucial technical capabilities that need development. The most important of these are: (1) Access to all of Mars–high and low latitudes, rough and smooth surfaces, low and high elevations, plus precision landing. (2) Access to the subsurface, from a meter to hundreds of meters, through a combination of drilling and geophysical sounding. (3) Access to time varying phenomena that requires the capability to make some measurements over a long period of time (e.g., climate studies covering from one to several Martian years). (4) Access to microscopic scales by instruments capable of measuring chemical and isotopic compositions and determining mineralogy and the nature of mineral intergrowths. Orbital and landed packages can make many of the high priority measurements, but others require that samples be returned from Mars. There is a strong consensus on the need for sample return missions. As noted in other MEPAG and National Academy of Science reports, study of samples collected from known locations on Mars and from sites whose geological context has been determined from remote sensing measurements has the potential to revolutionize our view of Mars. A full discussion of these issues is beyond the scope of this document, but we anticipate that they will be addressed by MEPAG and other scientific advisory committees in the near future.
Notes relating to this version of the Goals Document
The changes to this version of the Goals Document only involve relatively minor revisions and additions to Goals I-III. It is anticipated that a more extensive revision of the entire document will be undertaken as data from the Mars Reconnaissance Orbiter becomes available and is interpreted. Goal IV (Preparation for Human Exploration) was revised in 2005 with the assistance of a MEPAG chartered the Mars Human Precursor Science Steering Group (SSG) in order to clarify text in the 2001 and 2004 versions of the Goals Document regarding the time line and engineering implementation options for human missions to Mars.
The present version of the Goals Document incorporates changes made by the MEPAG Goals Committee and the broader science community. The Goals Committee provided comments and suggested revisions in late 2005 that were circulated to the MEPAG Executive Committee in December 2005. The entire document was then made available for comment and additional revisions by the science community in January 2006. All comments and additional revisions were then approved by the Goals Committee in January 2006 and the Executive Committee in February 2006.
I. GOAL: DETERMINE IF LIFE EVER AROSE ON MARS
Determining if life ever arose on Mars is a challenging goal. The focus of this goal is to determine if life is or was present on Mars. If life exists or existed, another focus is to understand the systems that support or supported it. Finally, if life never existed and conditions were suitable for formation and/or maintenance of life, a third focus would be to understand why evidence of life was not found. A comprehensive conclusion will necessitate understanding the planetary evolution of Mars and whether Mars is or could have been habitable, and will need to be based in multi-disciplinary scientific exploration at scales ranging from planetary to microscopic. The strategy we have adopted to pursue this goal has two sequential aspects: assess the habitability of Mars (which needs to be undertaken environment by environment); and, test for prebiotic processes, past life, or present life in environments that can be shown to have high habitability potential. These constitute two high-level scientific objectives. A critical means to achieve both of these objectives is to characterize Martian carbon chemistry and carbon cycling. Consequently, the science associated with carbon chemistry is so fundamental to the overall life goal that we have established it as a third primary science objective. To some degree, these overarching scientific objectives can be addressed simultaneously, as each requires basic knowledge of the distributions of water and carbon on Mars and an understanding of the processes that govern their interactions. Clearly, these objectives overlap, but are considered separately here.
To spur development of flight technologies required to achieve these objectives there must be an increase in the number of terrestrial ground truth tests for instrumentation on samples of relevance to Mars exploration. This aids the development of instrumentation directly while maintaining pressure to improve detection limits. In support of such tests, suites of highly characterized samples from relevant environments should be identified and curated for laboratory studies.
In order to prioritize the objectives and investigations described here, we need to be specific about the prioritization criteria. In broad perspective, Objective C (“test for life”) is a long-term goal. Objectives A (“assess habitability”) and B (“follow the carbon”) are the critical steps in narrowing the search space to allow Objective C to be addressed. We need to know where to look for life before making a serious attempt at testing for life. At the same time, Objectives A and B are fundamentally important even without searching for life directly; they help us understand the role planetary evolution plays in creating conditions in which life might have arisen, whether it arose or not. Thus, objectives A, B, and C, in this order, form a logical exploration sequence. Note that research goals and technology development plans must incorporate both short- and long-term scientific objectives.
A. Objective: Assess the past and present habitability of Mars (investigations listed in priority order)
As used in this document, the term “habitability” refers to the potential to support life of any form. Although Objective A is stated at a planetary scale, we know from our experience on Earth that we should expect that different environments on Mars will have different potential for habitability. It will not be possible to make measurements of one environment and assume that they apply to another. In order to address the overall goal of determining if life ever arose on Mars, the most relevant life detection investigations will be those carried out in environments that have high potential for habitability. Thus, understanding habitability in space and time is an important first order objective.
Arguably, until we discover an extant Martian life form and measure its life processes, there is no way to know definitively which combination of factors must simultaneously be present to constitute a Martian habitat. Until then, “habitability” will need to describe the potential of an environment to sustain life and will therefore be based on our understandings of habitable niches on Earth or plausible extrapolations. Current thinking is that at a minimum, the following three conditions need to be satisfied in order for an environment to have high potential for habitability:
- The presence of liquid water. As we currently understand life, water is an essential requirement. Its identification and mapping (particularly in the subsurface, where most of Mars’ water is thought to reside) can be pursued on a global, regional and local basis using established measurement techniques.
- The presence of the key elements that provide the raw materials to build cells
- A source of energy to support life.
Finally, environments with potential for habitability are assumed to have unequal potential to preserve the evidence in geological samples. There needs to be an understanding of these effects in order to understand the significance of many types of life-related investigations.
1. Investigation: Establish the current distribution of water in all its forms on Mars.
Water on Mars is thought to be present in a variety of forms and potential distributions, ranging from trace amounts of vapor in the atmosphere to substantial reservoirs of liquid, ice and hydrous minerals that may be present on or the below the surface. The presence of abundant water is supported by the existence of the Martian perennial polar caps, the geomorphic evidence of present day ground ice and past fluvial discharges, and by the Mars Odyssey GRS detection of abundant hydrogen (as water ice and/or hydrous minerals) within the upper meter of the surface in both hemispheres, at mid-latitudes and above. To investigate current habitability, the identity of the highest priority H2O targets, and the depth and geographic distribution of their most accessible occurrences, must be known with sufficient precision to guide the placement of subsequent investigations. To understand the conditions that gave rise to these potential habitats it is also desirable to characterize their geologic and climatic context. The highest priority H2O targets for the identification of potential habitats are: (1) liquid water — which may be present in as pockets of brine in the near-subsurface, in association with geothermally active regions (such as Tharsis and Elysium), as super-cooled thin films within the lower cryosphere, and beneath the cryosphere as confined, unconfined, and perched aquifers. (2) Massive ground ice – which may preserve evidence of former life and exist in a complex stratigraphy beneath the northern plains and the floors of Hellas, Argyre, and Valles Marineris, an expectation based on the possible former existence of a Noachian ocean, and the geomorphic evidence for extensive and repeated flooding by Hesperian-age outflow channel activity. (3) The polar layered deposits – whose strata may preserve evidence of climatically-responsive biological activity (at the poles and elsewhere on the planet) and whose ice-rich environment may result in episodic or persistent occurrences of liquid water associated with climate change, local geothermal activity and the presence of basal lakes.
2. Investigation: Determine the geological history of water on Mars, and model the processes that have caused water to move from one reservoir to another.
In order to assess past habitability, we need to start with understanding at global scale the abundance, form, and distribution of water in Mars’ geologic past. A first-order hypothesis to be tested is that Mars was at one time warmer and wetter than it is now. This can be done in part through investigation of geological deposits that have been affected by hydrological processes, and in part through construction of carefully conceived models. One key step is to characterize the regional and global sedimentary stratigraphy of Mars. It is entirely possible that Mars had life early in its history, but that life is now extinct.
3. Investigation: Identify and characterize phases containing C, H, O, N, P and S, including minerals, ices, and gases, and the fluxes of these elements between phases.
Assessing the availability of biological important elements and the phases in which they are contained, will allow a greater assessment of both habitability and the potential for life to have arisen. Detailed investigations for carbon are the primary focus of Objective B and therefore will not be further expounded upon here. Nitrogen, phosphorous and sulfur are critical elements for life (as they are on Earth), and the phases containing these elements and fluxes of these elements may reflect biological processes and the availability of these elements for life. They are often intimately associated with carbon and their distribution is commonly controlled by water and oxidation states, so interpreting these elemental cycles in terms of C, H, and O is extremely valuable to understanding habitability. The redox chemistry of S is of interest, because of its known role in some microbial metabolic strategies in terrestrial organisms and the abundance of sulfate on the surface of Mars.
4. Investigation: Determine the array of potential energy sources available on Mars to sustain biological processes.
This investigation would allow identification of the potential of Mars to have harbored or continue to harbor life. Biological systems require energy. Therefore, measurement of the availability of potential energy sources is a critical component of habitability, and understanding how life might use them is a critical component of designing scientifically robust life detection experiments. Sources of energy that should be measured may include chemical redox, pH gradients, geothermal heat, radioactivity, incident radiation (sunlight), and atmospheric processes.
B. Objective: Characterize Carbon Cycling in its Geochemical Context (investigations listed in priority order)
Carbon is the basic building block of life on Earth and is probably the building block of life on Mars (if life exists/existed). Understanding how carbon has been distributed on Mars through time, including now, is critical for understanding where to look for life on Mars, how life might have evolved on Mars, and how life might have originated on Mars. In addition, there may be aspects of the carbon cycle that reveal the existence of life (extant or extinct), and results are likely to strongly influence approaches to searching for other biosignatures. Thus, characterization of the carbon cycle is critical to determining if life ever arose on Mars.
Understanding the origin of organic carbon is particularly important and sources on Mars could be from several reservoirs that are summarized in Table 2. Once organic carbon is discovered, a major challenge will be in constraining the source of that organic carbon. Terrestrial contamination is a significant concern, because of the need to avoid false identification of organic carbon or specific organic molecules on Mars. In addition, meteoritic delivery of organic carbon to the surface of Mars and abiotic organic synthesis processes could produce measurable organic carbon concentrations. Understanding the origin of organic carbon is as important as identifying it.
We assume that extraterrestrial life would be based on carbon chemistry. Although this assumption may subsequently need to be relaxed, we would not know where else to begin in designing investigations of possible extraterrestrial life. If anomalous measurements indicate the presence of non-carbon based macromolecules associated with some form of life-like processes then further experiments can be designed to address this problem.
Terrestrial like organisms – from Earth Organisms not present on the craft measuring them, but had been previously transferred from Earth by either meteorite impact or contamination of previous spacecraft. Target molecules could include individual genes, membrane constituents, specific enzymes, and co-enzymes that would be expected to be over expressed or adapted in Martian conditions. Terrestrial-like organisms – evolved on Mars Organisms that utilize terrestrial like biochemistries and have evolved on Mars. Target molecules could include individual genes, membrane constituents, specific enzymes, and co-enzymes that would be expected to be over expressed or adapted in Martian conditions, or organisms using metabolisms that would not be present on a space craft contaminant such as methanogens, psychrophiles endolithic survival mechanisms. Non-terrestrial-like organisms Utilizes an array of molecules for information storage, information transfer, compartmentalization and enzymatic activity that differ from those used by extant terrestrial life. Examples would be the use of novel amino acids and nucleotides or the use of novel nitrogen utilization strategies. Fossil biomarkers Detection of established terrestrial fossil biomarkers such as hopanes, archaeal lipids and steranes, for the detection of the diagenetic remains of terrestrial based life.
1. Investigation: Determine the distribution and composition of organic carbon on Mars.
The spatial distribution and composition of organic carbon have not been characterized, but are instrumental in understanding the biological potential of Mars. (Methane and other simple reduced carbon molecules are included as “organic carbon” in this context.) Abiotic synthesis of organics, delivery of organics to Mars via meteorites, and possible biological production of organics must all be evaluated in the context of carbon cycling on Mars. Characterizing the molecular and isotopic composition of organic carbon is essential for determining the origin of the organics shown in Table 2, which includes the types of organic materials that need to be detected and deconvolved from each other. Investigations require sufficient spacecraft cleaning and verification to avoid likelihood of contamination, in addition to careful planning of specific methods to identify and exclude forward contamination at the experiment level. Example measurements include analysis of the concentration and isotopic composition of organic carbon, characterization of the molecular structure of organic carbon, or identifying and monitoring reduced carbon (e.g. methane) fluxes.
2. Investigation: Characterize the distribution and composition of inorganic carbon reservoirs on Mars through time.
Transformations of carbon between inorganic and organic carbon reservoirs are a characteristic of life. Evaluating carbon reservoirs and the fluxes among them is critical to understanding both the modern and geological evolution of carbon availability, and the inorganic carbon reservoirs are an important link in the cycle. The distribution of these reservoirs can also reveal critical habitability information because they can record climate records. Potential measurements include continued searching for carbonate minerals from orbit, in situ, and in returned samples, characterizing CO2 fluxes on various time scales globally and locally, and measuring the isotopic composition of any inorganic reservoir.
3. Investigation: Characterize links between C and H, O, N, P, and S
The carbon cycle is intimately linked to H, O, N, P, and S, particularly in the presence of life. Identifying connections among the geological cycles of these elements will substantially aid interpretations of the carbon cycle and may provide indicators that can be used to interpret biological potential. Potential measurements include mineralogical characterization of samples containing C, N, P, or S, isotopic and oxidation state characterization of S-containing phases, and identification of reactions involving any of these elements.
4. Investigation: Preservation of reduced compounds on the near-surface through time The surface of Mars is oxidizing, but the composition and properties of the responsible oxidant(s) are unknown. Characterizing the reactivity of the near surface of Mars, including atmospheric (e.g. electrical discharges) and radiation processes as well as chemical processes with depth in the regolith3 and within weathered rocks is critical to interpreting the paucity or possible absence of organic carbon on the surface of Mars. Understanding the oxidation chemistry and the processes controlling its variations will aid in predicting subsurface habitability if no organics are found on the surface. Potential measurements include identifying species and concentrations of oxidants, characterizing the processes forming and destroying them, and characterizing concentrations and fluxes of redox sensitive gases in the lower atmosphere.
C. Objective: Assess whether life is or was present on Mars (investigations listed in priority order)
This objective reflects several of NASA’s chief exploration goals. As mentioned earlier, the need to prevent false positives or negatives, and develop technology and experimental protocols, makes Objective C (“test for life”) a long-term goal. Objectives A (“assess habitability”) and B (“follow the carbon”) are the critical steps in narrowing the search space to allow Objective C to be addressed. Furthermore, the Objective itself does not halt upon a positive or negative answer. In the eventuality of a positive answer there would be the need to characterize whatever life form is discovered, as well as its origins and reason for surviving on Mars. In the case of a negative answer, then further characterization of why life did not start on Mars would become a priority and in itself help us to understand more about life on earth.
Determining whether life ever existed on Mars is a scientifically exciting and challenging endeavor. The following investigations look for biosignatures, which are defined as results that REQUIRE the presence or past presence of life. Commonly, multiple observations in a context are required to identify biosignatures, and multiple scales of observation are very important. Four investigations of features currently recognized as biosignatures are listed here.
3 Regolith. As used in this document, a general term partially cohesive material, of any origin (in-situ, surface of Mars (after Glossary of Geology).
Investigation 1 (Characterize complex organics) is considered to be the highest priority.
Investigation 1 and some measurements to address Investigation 4 require sufficient spacecraft cleaning and verification to avoid likelihood of contamination, in addition to careful planning of specific methods to identify and exclude forward contamination at the experiment level. Investigations 2 and 3, which depend on the spatial distribution of signatures, are less sensitive to contamination and may be more practical to pursue first. Remote sensing techniques addressing investigation 4 also have much lower to no contamination issues. Investigations 1-4 are largely in situ investigations that are best conducted in those habitable environments identified in A1. A fifth investigation concept consists of suites of observations based on correlations in biological indicators, which by themselves are only suggestive for life and only in combination can provide a true biosignature. It seems likely that many of the combinations of measurements have yet to be identified, and it is expected that exciting proposals for suites of observations will be seriously considered in choosing investigations to evaluate the past or present presence of life.
1. Investigation: Characterize complex organics.
The identification of complex organics that can only be produced biologically is a very strong biosignature, if forward contamination by terrestrial organics can be excluded. Measurements for this investigation must include appropriate methods to identify and exclude forward contamination as a source of the target materials. To this end new instruments must be developed for cleaning and monitoring of spacecraft contamination. Instruments must be required to produce procedural blanks that allow accurate measurements by that instrument to be undertaken. This entails that the critical path of contamination, i.e. the path the sample takes to the instrument, be cleaned to a level below the detection limit of the instrument. Example measurements may include characterization of organics such as DNA, nucleotides, chlorophyll, etc. for extant life; hopanes, steranes, isoprenoids, etc. for fossil life; or cumulative properties and/or distributions of organics such as homochirality.
2. Investigation: Characterize the spatial distribution of chemical and/or isotopic signatures.
The spatial distribution of chemical or isotopic variations can be a biosignature, if the distribution is inconsistent with abiotic processes. Example measurements may include imaging of the distribution of organics on a surface or in minerals; identifying correlations among isotopic values and elemental concentrations that reflect biological processes; or the presence of reduced and oxidized gas phases in disequilibrium.
3. Investigation: Characterize the morphology or morphological distribution of mineralogical signatures.
Sedimentary and weathered rocks can preserve biosignatures in the distribution of grains and minerals or in the morphology of biologically produced minerals. Example measurements may include micron to nanometer imaging and chemical analysis of crystals or morphological characterization of sedimentary lamination to regional or global scale characterization of sedimentary stratigraphy.
4. Investigation: Identify temporal chemical variations requiring life.
Extant life may be active, producing observable temporal changes in chemistry over the time scale in which a lander experiment may be functional. Monitoring systems that may harbor life is an excellent way to identify the presence of life. However, possible abiotic reactions need to be thoroughly understood and forward contamination needs to be identified or excluded. It is critical that measurements capable of being contaminated include appropriate methods to identify and exclude forward contamination as a source of the signatures being monitored. Example measurements may include monitoring the flux of gases thought to be biologically produced; monitoring oxidative changes in a way that excludes abiotic reactions; or performing experiments to look for metabolic processes.
II. GOAL: UNDERSTANDING THE PROCESSES AND HISTORY OF CLIMATE ON MARS
The fundamental scientific questions that underlie this goal are how the climate of Mars has evolved over time to reach its current state, and what processes have operated to produce this evolution. These extremely important scientific questions are in accord with several key science objectives found in the NASA Solar System Exploration Roadmap (2003). Mars climate can be defined as the mean state and variability of its atmosphere and exchangeable volatile reservoirs (near the surface) evaluated from diurnal to geologic time scales. An understanding of Mars climatic evolution rests upon gaining a full understanding of the fundamental processes governing its climate system, and thus upon obtaining detailed observations of the current (observable) system. Goal II also is in line with the recent recommendation of the Solar System Exploration Survey [2002], which calls out the clear need for Mars upper atmosphere measurements to properly characterize current volatile escape rates. The Objectives below are given in priority order. Objective A is crucial to understanding the present state of the entire atmospheric system (from the surface-atmosphere boundary to the exosphere). It forms the baseline for interpreting past climate on Mars. Objective B focuses upon specific investigations that will measure key indicators of the past climate of Mars. Finally, Objective C highlights mission critical atmospheric measurements that will reduce mission risk and enhance overall science return, benefiting all future missions to the planet. No attempt has been made to prioritize these risk mitigation and engineering related measurements since all are important.
A. Objective: Characterize Mars’ Atmosphere, Present Climate, and Climate Processes (investigations in priority order)
Our understanding of the composition and dynamics of the present Martian atmosphere is the basis for understanding past climate on Mars. Investigations of both the upper and lower atmosphere are essential because they are one large, interconnected system. Measurements of both atmospheric regions enable us to explore different suites of processes that play unique roles in understanding the Martian climate and its evolution. In short, a ground-to-exosphere approach to monitoring the Martian atmospheric structure and dynamics is needed for a proper characterization of the present day climate of Mars.
1. Investigation: Determine the processes controlling the present distributions of water, carbon dioxide, and dust by determining the short and long-term trends (daily, seasonal and solar cycle) in the present climate. Determine the present state of the upper atmosphere (neutral/plasma) structure and dynamics; quantify the processes that link the Mars lower and upper atmospheres.
To understand the present climate system, from the surface to the exosphere, requires long-term (multi-year) continuous global monitoring from both landed and orbital platforms. Understanding the factors that control the annual variations of volatiles and dust is necessary to determine to what extent processes operating today have controlled climate change in the past.
(i) Lower atmosphere climate and processes
Landed missions can provide direct in situ measurements and are the best way to measure near-surface water vapor, winds, and other variables that control the exchange of volatiles and dust between the surface and atmosphere.
Orbital missions can provide information on the global and vertical structure of the atmosphere, direct measurement of winds, and information on the spatial distribution of aerosols, water vapor, and potentially other important trace species. This information leads to the elucidation of the local through global scale processes that operate to maintain the climate and transport volatiles and dust. The global meteorological, radiative, and mass balance observations gathered from these platforms on daily- to decade-long timescales will establish the magnitude of inter-annual variability, aid in the identification of the responsible mechanisms, and demonstrate whether there are any long-term trends in the present climate system. These observations will also assist in identifying the causes of the north/south asymmetry in the nature of the polar caps, and the physical characteristics of the layered deposits. These data will serve as the foundation for the development of more realistic models to assess the effects of various external forcing-factors (such as obliquity and increased atmospheric pressure) on the climate of Mars.
(ii) Upper atmosphere climate and processes
Orbiter missions are also needed to investigate the mean state and variability of the neutral and plasma environment above ~80 km. These data will improve our understanding of the coupling of the Martian lower and upper atmospheres, and characterize the regions of the upper atmosphere that interact with the solar wind. Also, the global characterization of the present lower and upper atmosphere structure and dynamics is required over various timescales (daily, seasonal, and solar cycle) in order to properly interpret volatile escape measurements and the subsequent volatile evolution model results. This reemphasizes the need for a ground-to-exosphere approach to monitoring the Martian atmospheric structure and dynamics.
2. Investigation: Search for microclimates.
Detection of exceptionally or recently wet or warm locales, exceptionally cold localities, and areas of significant change in surface accumulations of volatiles or dust would identify sites for in situ exploration. This requires a global search for sites based on local surface properties (e.g., geomorphic evidence, topography, local thermal properties, albedo) or changes in volatile (especially H2O) distributions.
3. Investigation: Determine the production/loss, reaction rates, and global 3-dimensional distributions of key photochemical species (O3, H2O2, CO, OH, CH4, SO2, etc.), and their interaction with surface materials.
This investigation necessarily involves study of both the lower and upper atmosphere. Surface sinks and sources and lower atmospheric distributions are required to interpret atmospheric escape rates and upper atmosphere aeronomic processes. Current multi-dimensional photochemical models predict global distributions of these species. Such models require validation to confirm key reactions and rates and the role of dynamics in the transport of these constituents. There is, however, considerable uncertainty over surface fluxes of major species. This investigation requires global orbiter observations of neutral and ion species, temperatures, and winds in the lower and upper atmospheres, and the systematic monitoring of these atmospheric fields over multiple Mars years to capture inter-annual variability induced by the solar cycle, seasons, and dust storms.
B. Objective: Characterize Mars’ Ancient Climate and Climate Processes Through Study of the Geologic and Volatile Record of Climate Change (investigations in priority order)
Understanding the ancient climate and climate processes on Mars requires interdisciplinary study of the Martian surface and atmosphere. The investigations described below focus on quantitative measurements (concentrations and isotopic compositions) of important atmospheric gases in the atmosphere and trapped in surface materials. It also requires study of geologic features to search for the record of past climates.
1. Investigation: Determine the stable isotopic, noble gas, and trace gas composition of the present-day bulk atmosphere.
These provide quantitative constraints on the evolution of atmospheric composition and on the sources and sinks of the major gas inventories. It is important to understand the temporal and spatial variability of atmospheric composition. This investigation requires high-precision isotopic measurements of the atmosphere.
2. Investigation: Determine the rates of escape of key species from the Martian atmosphere, their correlation with seasonal and solar variability, their modification by remnant crustal magnetic fields, and their connection with lower atmosphere phenomenon (e.g. dust storms). From these observations, quantify the relative importance of processes that control the solar wind interaction with the Mars upper atmosphere in order to establish the magnitude of associated volatile escape rates.
These measurements will provide crucial constraints to atmospheric evolution models that extrapolate these rates to determine past climates. This investigation requires global orbiter observations of neutral and plasma species, crustal magnetic fields, temperatures, and winds in the extended upper atmosphere. The systematic monitoring of these fields over multiple Mars years is needed to capture the inter-annual variability induced by the solar cycle, seasons, and dust storms. This investigation also requires more thorough and higher-resolution measurements of crustal magnetic fields.
3. Investigation: Determine how the stable isotopic, noble gas, and trace gas composition of the Martian atmosphere has evolved through time.
These provide quantitative constraints on the evolution of atmospheric composition and on the source and sinks of water and other major gas inventories. Requires high precision dating and isotopic measurements of Martian meteorites and returned samples, and high precision in situ measurements of samples on and beneath the surface (e.g., polar layered-deposits or strata exposed in Valles Marineris).
4. Investigation: Find physical and chemical records of past climates.
This investigation centers on finding geomorphic and chemical evidence of past climates or of prior environmental events or conditions that may have perturbed the local or global climate in unexpected ways (e.g., the former presence of an ocean or seas, large impacts, episodic volcanism or outflow channel activity). These provide the basis for understanding the extent, duration (e.g., gradual change or abrupt transition), and timing of past climates on Mars. This investigation requires determining sedimentary stratigraphy and the distribution of aqueous weathering products.
5. Investigation: Characterize the stratigraphic record of climate change preserved in polar layered deposits, residual ice caps, other climate-modulated deposits of H2O, CO2, and dust found elsewhere on the planet.
The presence of extensive layered deposits suggests that the climate of Mars has undergone frequent and geologically recent change. A key to understanding the climatic and geologic record preserved in these deposits is to determine (i) the relative and absolute ages of the layers, (ii) their thickness, extent and continuity, (iii) their petrologic/geochemical characteristics (including both isotopic and chemical composition), and (iv) the environmental conditions and processes that were necessary to produce them. Specific examples of the type of information these deposits may preserve include a stratigraphic record of volatile mass balance; insolation variations; atmospheric composition; dust storm, volcanic and impact activity; cosmic dust; catastrophic floods; solar luminosity (extracted by comparisons with terrestrial ice cores); supernovae and perhaps even a record of microbial life. Addressing this investigation requires high-resolution imaging, in situ and remote sensing measurements of stratigraphy and layer properties, and absolute ages determined either in situ or from returned samples.
C. Objective: Characterize the State and Processes of the Martian Atmosphere of Critical Importance for the Safe Operation of Spacecraft (no priority order).
This objective focuses on atmospheric processes of importance for the safe implementation of spacecraft missions. These investigations will yield the critical information necessary to improve the likelihood of successful execution of missions in the Martian environment. Investigations seek to characterize the atmosphere from the surface to 400 km altitude to support spacecraft landing, flight, aerocapture, aerobraking, long-term orbital stability, targeting of observations from orbit, and mission planning. Every effort should be made to accommodate instruments that address these investigations on each spacecraft bound for Mars.
1. Investigation: Understand the thermal and dynamical behavior of the planetary boundary layer.
The lowest portion (<5km) of the atmosphere can be highly turbulent. Horizontal and vertical winds in this region represent a significant risk to spacecraft Entry, Descent, and Landing (EDL) and the operation of aerial platforms (balloons and aeroplanes). The turbulence also transports heat, so is a concern for thermal design. The turbulence is driven by thermal contrasts between the surface and the atmosphere, and mechanical interactions between the mean wind and the rough planetary surface. This investigation is designed to probe the connections between surface temperature, the modification of surface and air temperatures by aerosol radiative heating, and the dynamical and thermal state of the lower atmosphere.
2. Investigation: Understand and monitor the behavior of the lower atmosphere (0-80km) on synoptic scales.
Mars exhibits significant seasonal and dramatic, episodic changes in the state of the atmosphere. Of great concern to the operation of surface and near-surface spacecraft, and for aerobraking, aerocapture, or aeropass maneuvers, is the onset of regional and global dust storms. The mechanisms of storm development are unknown at this time, and thus current models cannot predict them. Prediction will require much further study of these events, while early observation will greatly mitigate them. It is critical for support of Mars missions that continuously-operational, meteorological assets be maintained in Martian orbit to improve our understanding of the processes so that we can better predict atmospheric conditions at the time a spacecraft arrives and to make observations near the time of entry.
3. Investigation: Determine the atmospheric mass density and its variation over the 80 to 200 km altitude range.
Aerobraking, aerocapture, and aeropass operations use the atmosphere as a brake. These operations are safest when the drag imparted by the atmosphere can be accurately estimated ahead of time. Unfortunately, the Martian atmosphere exhibits substantial variations in density at a given geometric height due to the influence of the diurnal cycle, seasonal cycle, large-scale atmospheric circulation, and the propagation of waves. Mapping and understanding the processes responsible for the density variations in the upper atmosphere is critical to high-precision spacecraft trajectory planning. For example, techniques for measuring and predicting the mass density on time scales of hours to days are key requirements for aerobraking. This information is also important for mission planning (e.g., estimating the amount of fuel needed throughout mission life).
4. Investigation: Determine the atmospheric mass density and its variations at altitudes above 200 km.
The constraint of mass density variations at high altitudes is important for precision targeting of orbital observations and for long-term orbital stability required to meet planetary protection mandates.
III. GOAL: DETERMINE THE EVOLUTION OF THE SURFACE AND INTERIOR OF MARS
Insight into the composition, structure, and history of Mars is fundamental to understanding the solar system as a whole, as well as providing insight into the history and processes of our own planet. Thus there are compelling scientific motivations for the study of the surface and interior of the planet in its own right. The recent heightened interest in the possibility of life on Mars provides additional emphasis for these investigations. Geology informs virtually every aspect of the study of conditions conducive to the origin and persistence of life, and the study of the interior provides important clues about a wide range of topics, including the early history of Mars, sources of volatiles, and geothermal energy.
The unique aspect of Mars, which in many ways make it appear more Earth-like and sets it apart from the other planet, is evidence of the presence and activity of liquid water at or near the surface. This has enormous geological implications affecting, for example, erosion, weathering, heat flow, and the possibility of life (which can, in turn, have significant effects on geological processes). Thus, an emphasis on water is a logical framework within which to explore the planet.
A. Objective: Determine the nature and evolution of the geologic processes that have created and modified the Martian crust and surface (investigations in priority order)
The Martian surface contains the record of all the processes that shaped it, from initial differentiation to volcanism, to modification by impact, wind, and water. Understanding that record will help us understand the total inventory and role of water on Mars, regions likely to have been habitable to life, the processes involved in surface-atmosphere interactions, and the thermal history of the planet.
1. Investigation: Determine the present state, 3-dimensional distribution, and cycling of water on Mars.
Water is an important geologic material on Mars, influencing most geological processes including the formation of sedimentary, igneous and metamorphic rocks, the weathering of geological materials, and deformation of the lithosphere. Determining the distribution of water on Mars requires global observations using subsurface sounding and remote sensing, coupled with detailed local and regional sounding and measurements.
2. Investigation: Evaluate fluvial, subaqueous, pyroclastic, subaerial, and other sedimentary processes and their evolution and distribution through time, up to and including the present.
Fluvial and lacustrine sediments are likely sites to detect traces of prebiotic compounds and evidence of life. Sediments also record the history of water processes on Mars. Polar layered terrains in particular may preserve a unique record of climate history. Aeolian sediments record a combination of globally averaged and locally derived fine-grained sediments and weathering products. Understanding sedimentary processes requires knowledge of the age, sequence, lithology, and composition of sedimentary rocks (including chemical deposits, such as those observed at Meridiani); the rates, durations, environmental conditions, and mechanics of weathering, cementation, and transport processes; and the fluvial and lacustrine record preserved in the morphology of the surface and shallow subsurface (e.g., beneath surficial deposits).
3. Investigation: Calibrate the cratering record and absolute ages for Mars.
The evolution of the surface, interior, and surface of Mars, as well as the possible evolution of life, must be placed in an absolute timescale, which is presently lacking for Mars. Developing this chronology requires determining the absolute ages of geological units of known crater ages.
4. Investigation: Evaluate igneous processes and their evolution through time, including the present.
This investigation includes the broad range of igneous processes including, for example, volcanic outgassing and volatile evolution. In addition to dramatically molding the surface of the planet, volcanic processes are the primary mechanism for release of water and atmospheric gasses that support potential past and present life and human exploration. Sites of present day volcanism, if any, may be prime sites to investigate for life. This investigation requires global imaging at a range of wavelengths, geologic mapping, in-situ and remote techniques for distinguishing igneous and sedimentary rocks, evaluation of current activity.
5. Investigation: Characterize surface-atmosphere interactions on Mars, including polar, Aeolian, chemical, weathering, mass-wasting and other processes.
The focus of this investigation is on processes that have operated for the last million years as recorded in the upper 1 m to 1 km of Mars. Understanding present geologic, hydrologic, and atmospheric processes is the key to understanding past environments and possible locations for near-surface water. Knowing the morphology, chemistry and mineralogy of both near surface rocks and alteration products is essential for calibrating remote sensing data. This study also has strong implications for resources and hazards for future human exploration. It requires orbital and surface-based remote sensing of the surface and shallow subsurface (meters to 10s of meters), and direct measurements of sediments and atmospheric boundary layer processes.
6. Investigation: Determine the large-scale vertical structure and chemical and mineralogical composition of the crust and its regional variations. This includes, for example, the structure and origin of hemispheric dichotomy.
The vertical and global variation of rock properties and composition record formative events in the planet’s early history constrain the distribution of subsurface aquifers, and aid interpretation of past igneous and sedimentary processes. Determining these structures requires global and local remote sensing and deeper subsurface sounding (100s of meters to kilometers), detailed geologic mapping, and determination of mineralogy and composition of surface material.
7. Investigation: Document the tectonic history of the Martian crust, including present activity.
Understanding the tectonic record is crucial for understanding the geologic history as well as the temporal evolution of internal processes. This, in turn, places constraints on release of volatiles from differentiation and volcanic activity and the effect of tectonic structures (faults and fractures in particular) on subsurface hydrology. This investigation requires geologic mapping using high resolution topographic data combined with high-resolution images at a range of wavelengths, magnetic and gravity data, and seismic monitoring.
8. Investigation: Evaluate the distribution and intensity of hydrothermal processes through time, up to and including the present.
Hydrothermal systems are thought to be the connected with the earliest evolution of life on the Earth. Hydrothermal systems may also play an important role in the chemical and isotopic evolution of the atmosphere, and the formation of the Martian regolith. Deposits from hydrothermal systems have the potential to record the history of the biosphere and crust-atmosphere interactions throughout Martian history. Assessing hydrothermal processes requires knowledge of the age and duration of the hydrothermal system, the heat source, and the isotopic and trace element chemistry and mineralogy of the materials deposited.
9. Investigation: Determine the processes of regolith formation and subsequent modification, including weathering and diagenetic processes.
The regolith is a filter through which we view most of the Martian surface by remote sensing. In addition, it may provide a valuable record of the history of surface conditions and processes. Understanding regolith formation and modification requires quantitative measurement of mineralogy, chemistry, and physical parameters of the surface and shallow subsurface.
10. Investigation: Determine the nature of crustal magnetization and its origin.
The magnetization of the Martian crust is poorly understood, but is intimately related to the igneous, thermal, tectonic and hydrologic history of the crust. Addressing this problem requires high-resolution mapping of the magnetic field and knowledge of the mineralogy and magnetization of the surface.
11. Investigation: Evaluate the effect of impacts on the evolution of the Martian crust.
Impact is arguably the most important of the processes shaping the crust and surface of Mars. A firm understanding of effects of impacts on the structural, topographic and thermal history is a prerequisite for any broad understanding of the Martian crust and surface. Understanding impact effects requires geologic mapping using global topographic data combined with high-resolution images and remote sensing.
B. Objective: Characterize the structure, composition, dynamics, and evolution of Mars’ interior (investigations in priority order)
Investigating the internal dynamics and structure of Mars contributes to understanding the bulk chemical composition of Mars, the evolution of its crust and mantle, and the origin of its magnetic field and the nature and origin of the minerals that record the field. These are fundamental aspects of Mars that for the basis of comparative planetology.
1. Investigation: Characterize the structure and dynamics of Mars’ interior.
Understanding the structure and dynamical processes of the deep interior is fundamental for understanding the origin and evolution of Mars in general, and its surface evolution and the release of water and atmospheric gasses in particular. For example, the thickness of the crust and the size of the core provide strong constraints on the bulk composition of the planet and the manner in which it differentiated. This investigation requires mineralogic, isotopic, seismic, magnetic, gravity and heat flow data bearing directly and indirectly on interior structure and processes.
2. Investigation: Determine the origin and history of the magnetic field.
Evidence that Mars had a magnetic field early in its history has important implications for its formation and early evolution, as well as for the retention of its early atmosphere and for the shielding of the surface from incoming radiation and the possible evolution of life. Requires high-precision, high-resolution global, regional, and local magnetic measurements, as well as mineralogic, isotopic, seismic, gravity and heat flow data bearing on interior structure and processes.
3. Investigation: Determine the chemical and thermal evolution of the planet.
Knowledge of the chemical and thermal evolution places constraints on the composition, quantity, and rate of release of volatiles (water and atmospheric gasses) to the surface. This investigation requires measurements of the internal structure, thermal state, surface composition and mineralogy, and geologic relationships.
4. Investigation: Study the structure, dynamics and composition of Phobos and Deimos as indicators of the formation and early evolution of Mars.
Dynamical arguments suggest that the origin of Phobos and Deimos are intimately connected with that of Mars itself. The dynamics of their orbits may provide constraints on the structure of Mars’ interior and their composition may provide further clues to its evolution. This investigation is not aimed at detailed studies of Phobos and Deimos, but rather at using their properties to constrain the composition and evolution of Mars.
GOAL IV: PREPARE FOR HUMAN EXPLORATION
Robotic missions serve as logical precursors to eventual human exploration of space. In the same way that the Lunar Orbiters, Ranger and Surveyor landers paved the way for the Apollo moon landings, a series of robotic Mars Exploration Program missions is charting the course for future human exploration of Mars. Goal IV of the MEPAG document differs from the previous Goals in that it addresses science and engineering questions specific to increasing the safety, decreasing the cost, and increasing the productivity of human crews on Mars. To address these issues this section describes both the data sets that are to be collected and analyzed (Objective A), and the demonstrations of critical technologies that must be validated in the actual Martian environment (Objective B).
The 2004 National Vision for Space Exploration provides guidance for a broad range of human and robotic missions to the moon, Mars and destinations beyond. Robotic missions serve as one component of a “system of systems”, the sum of which work together to accomplish the goals of implementing a safe, sustained, and affordable robotic and human program to explore and extend human presence across the solar system and beyond. One of the Vision’s main points is to conduct robotic exploration of Mars to prepare for human exploration, and NASA has adopted a “level zero” requirement to “conduct robotic exploration of Mars to search for evidence of life, to understand the history of the solar system, and to support future human exploration activities.” Specifically, robotic precursor missions will be used in part to acquire and analyze data for the purpose of reducing cost and risk of future human exploration missions, to perform technology and flight system demonstrations for the purpose of reducing cost and risk of future human exploration missions, and to deploy infrastructure to support future human exploration activities.
As part of the momentum associated with the 2004 National Vision for Space Exploration, in 2004-2005 MEPAG undertook a major reassessment of the issues associated with preparing for the human exploration of Mars. This work is summarized in the following two documents, which represent analyses of Goal IV Objective A, and Goal IV Objective B, respectively. The logic associated with the investigations and measurements is described in these reports.
Beaty, D.W., Snook, K., Allen, C.C., Eppler, D., Farrell, W.M., Heldmann, J., Metzger, P., Peach, L., Wagner, S.A., and Zeitlin, C., (2005). An Analysis of the Precursor Measurements of Mars Needed to Reduce the Risk of the First Human Missions to Mars. Unpublished white paper, 77 p, posted June 2005 by the Mars Exploration Program Analysis Group (MEPAG) at http://mepag.jpl.nasa.gov/reports/index.html.
Hinners, N.W., Braun, R.D., Joosten, K.B., Kohlhase, C.E., and Powell, R.W., (2005), Report of the MEPAG Mars Human Precursor Science Steering Group Technology Demonstration and Infrastructure Emplacement (TI) Sub-Group, 24 p. document posted July, 2005 by the Mars Exploration Program Analysis Group (MEPAG) at http://mepag.jpl.nasa.gov/reports/index.html.
Objective A. Obtain knowledge of Mars sufficient to design and implement a human mission with acceptable cost, risk and performance.
Investigations #1A-1D are judged to be of indistinguishable high priority. Investigation 1A. Characterize the particulates that could be transported to hardware and infrastructure through the air (including both natural Aeolian dust and other materials that could be raised from the martian regolith by ground operations), and that could affect engineering performance and in situ lifetime. Analytic fidelity sufficient to establish credible engineering simulation labs and/or performance prediction/design codes on Earth is required. Measurements
a. A complete analysis, consisting of shape and size distribution, mineralogy, electrical and thermal conductivity, triboelectric and photoemission properties, and chemistry (especially chemistry of relevance to predicting corrosion effects), of samples of regolith from a depth as large as might be affected by human surface operations.
Note #1: For sites where air-borne dust naturally settles, a bulk regolith sample is sufficient—analysis of a separate sample of dust filtered from the atmosphere is desirable, but not required.
Note #2: Obtaining a broad range of measurements on the same sample is considerably more valuable than a few measurements on each of several samples (this naturally lends itself to sample return).
Note #3: There is not consensus on adding magnetic properties to the list of measurements.
b. Characterize at least one regolith deposit with fidelity sufficient to establish credible engineering simulation labs and/or software codes on Earth to solve engineering problems related to differential settlement of the regolith, and plume/regolith interactions (see Note #4).
1. For one site on Mars (see Note #5), measure the following properties of the regolith as a function of depth to 1 meter:
(i) Particle shape and size distribution
(ii) Ice content and composition to within 5% by mass
(iii) Regolith density to within 0.1 g/cm3
(iv) Gas permeability in the range 1 to 300 Darcy with a factor of three accuracy.
(v) Presence of significant heterogeneities or subsurface features of layering
(vi) An index of shear strength
(vii) Flow Rate Index test or other standard flow index measurement
2. Repeat the above measurements at a second site in different geologic terrane:
c. The same measurements as in a) on a sample of air-borne dust collected during a major dust storm.
d. Subsets of the complete analysis described in a), and measured at different locations on Mars (see Note #2). For individual measurements, priorities are:
i. shape and size distribution and mineralogy
ii. electrical
iii. Chemistry
4 General Notes:
? Except as noted, investigations are listed in priority order. Within each investigation, measurements are listed in priority order.
? Prioritization Criteria: o Magnitude of effect of precursor information on reduction of risk and/or cost of a human mission to Mars.
o Perceived degree of viability and cost of available mitigation options.
o Potential to obtain minimum necessary information in a less expensive way than by flying a mission to Mars.
? Assumptions: o The first human mission includes a landed human element.
o The possibilities of “short-stay” (~30 sols) and “long-stay” (~300 sols) are both under consideration, so the precursor program needs to support both.
Note #4. Because there is a large engineering lead-time required to solve the geotechnical problems, these data must be obtained early in the precursor program.
Note #5. These measurements should be made in a competent regolith deposit as opposed to loose drift material (cohesionless sand dunes), as landing is expected to attempt to avoid the looser material. Also, if mission planners select high latitude polar deposits for a human landing site, geotechnical data will be required from a representative location of those deposits. These measurements should include polarity and magnitude of charge on individual dust particles suspended in the atmosphere and concentration of free atmospheric ions with positive and negative polarities. Measurement should be taken during the day in calm conditions representative of nominal Extra Vehicular Activity (EVA) excursions.
Investigation 1B. Determine the atmospheric fluid variations from ground to >90 km that affect EDL and TAO including both ambient conditions and dust storms.
Measurements:
a. Measure v, P, T and _ in the upper, middle and lower atmosphere during EDL. Obtain as many profiles at various times and locations as possible (requested for ALL landed missions). Sample rate should be high enough (~ 100 Hz) to quantify turbulent layers. Specific direct or derived measurements include:
- Density from 120 km to surface ranging from high altitude values of 10-9 to near-surface values of 10-1 kg/m3, d_ = 1% of local ambient, rate= 100 Hz
- Pressure from 120 km to surface ranging from high altitude values of 10-7 to near-surface values of 15 mb, dP= 1% of local ambient, rate =100 Hz
- Temperature 60-300 K, dT = 0.5K, rate= 100 Hz (direct measurement may be slower)
- Directional Wind Velocity, 1-50 m/sec, dv = 1 m/s, rate= 100 Hz
- Particular emphasis on measurements between 0-20 km to quantify boundary layer wind and turbulence and 30-60 km where vehicle dynamic pressure is large.
b. Monitor surface/near-surface v(z), P, T(z), and _ as a function of time. Quantify the nature of the surface heating driver and associated boundary layer turbulence at altitudes above station. Data defines the initial conditions for high altitude modeling. Obtain data from as many locations as possible (requested for all landed missions). Surface/near surface packages should measure directly:
- Pressure, at surface, 0.005 mb to 15 mb, dP = 2 microb, full diurnal sampling, rate= >10 Hz
- Velocity, at surface, 0.05-50 m/sec, dv = 0.05 m/s, horizontal and vertical, full diurnal sampling, rate= 10 Hz
- Air temperature, at surface, 150-300 K, dt = 0.04K, full diurnal sampling, rate= 10 Hz
- Ground temperature 150-300 K, dt = 1K, full diurnal sampling, rate= 1 Hz
- Air temperature profile, 0-5km, <1km resolution, 150-300K, dt=2K, full diurnal sampling, rate=1 Hz
- Velocity profile, 0-5km, <1km resolution, 1-50 m/sec, dv=1 ms/, horizontal and vertical, full diurnal sampling, rate=1 Hz
o Opacity, visible, depth 0.2-10, dtau = 0.1, once every 10 min/li>
c. Make long-term (>> 1 martian year) remote sensing observations of the weather (atmospheric state and variations) from orbit, including a direct or derived measurement of:
- Aeolian, cloud, and fog event frequency, size, distribution as a function of time, over multi-year baseline.
- Vertical temperature profiles from 0-120 km with better than 1 km resolution between 0-20 km, 1-3 km resolution between 20-60 km, 3 km resolution > 60 km and with global coverage over the course of a sol, all local times [Development work required for T from surface to 20 km].
- Vertical density/pressure profiles from 0-120 km with better than 1 km resolution between 0-20 km, 1-3 km resolution between 20-60 km, 3 km resolution > 60 km and with global coverage over the course of a sol, all local times [Development work required for _ from surface to 20 km].
- 3-D winds as a function of altitude, from 0-60 km with better than 1 km resolution below 20 km, and 1-3 km resolution between 20-60 km, and with global coverage over the course of a sol, all local times [Development work required at all altitudes for an independent means to derive V, with special emphasis from surface to 20 km].
Note particular emphasis on measurements between 0-20 km to quantify boundary layer wind and turbulence and 30-60 km where vehicle dynamic pressure is large.
d. At time of human EDL and TAO, deploy ascent/descent probes into atmosphere to measure P, V, and T just prior to human descent at scales listed in 1Ba.
Note #6: We have not reached agreement on the minimum number of atmospheric measurements described above, but it would be prudent to instrument all Mars atmospheric flight missions to extract required vehicle design and environment information. Our current understanding of the atmosphere comes primarily from orbital measurements, a small number of surface meteorology stations and a few entry profiles. Each landed mission to Mars has the potential to gather data that will significantly improve our models of the martian atmosphere and its variability. It is thus desired that each opportunity be used to its fullest potential to gather atmospheric data. Reconstructing atmospheric dynamics from tracking data is useful but insufficient. Properly instrumenting entry vehicles is required.
1C. Determine if each martian site to be visited by humans is free, to within acceptable risk standards, of biohazards that may have adverse effects on humans and other terrestrial species. Sampling into the subsurface for this investigation must extend to the maximum depth to which the human mission may come into contact with uncontained martian material. Measurements:
a. Determine if extant life is widely present in the martian near-surface regolith, and if the air-borne dust is a vector for its transport. If life is present, assess whether it is a biohazard. For both assessments, the required measurements are the tests described in the Draft Test Protocol.
Note #7: To achieve the necessary confidence, this requires sample return and analyses in terrestrial laboratories.
Note #8: The samples can be collected from any site on Mars that is subjected to wind-blown dust.
Note #9: At any site where dust from the atmosphere is deposited on the surface, a regolith sample collected from the upper surface is sufficient–it is not necessary to filter dust from the atmosphere.
b. At the site of the planned first human landing, conduct biologic assays using in-situ methods, with measurements and instruments designed using the results of all prior investigations. All of the geological materials with which the humans and/or the flight elements that will be returning to Earth come into contact need to be sampled and analyzed.
Note #10: It is recommended that a decision on whether human landing sites after the first one require a lander with biological screening abilities be deferred until after Measurement a) has been completed.
Investigation 1D. Characterize potential sources of water to support In Situ Resource Utilization (ISRU) for eventual human missions. At this time it is not known where human exploration of Mars may occur. However, if ISRU is determined to be required for reasons of mission affordability and/or safety, then the following measurements for water with respect to ISRU become necessary (these options cannot be prioritized without applying constraints from mission system engineering, ISRU process engineering, and geological potential):
Measurement Options:
a. Perform measurements within the top few meters of the regolith in a location within the near-equatorial region (approximately ±30°) that the Mars Odyssey mission indicates is a local maximum in hydrogen content, to determine: (i) concentration of water released upon regolith heating, (ii) composition and concentration of other associated volatiles released with water, and (iii) three-dimensional distribution of measurements i & ii within a 100 meter x 100 meter local region. This option would include water contained in hydrous minerals, as adsorbed water, and in any other form it might be present in the regolith. Either unconsolidated or loosely consolidated regolith is a focus of current attention because of the need to minimize mining engineering, but outcrops of rock containing hydrous minerals may also be a valuable possibility if they are sufficiently friable.
b. Perform measurements to (i) identify and determine the depth, thickness, and concentration of water in subsurface ice deposits to a few meters depth at approximately 40° to 55° latitude, (ii) determine the demarcation profile/latitude where near-surface subsurface ice formation does and does not occur.
c. Perform measurements in the polar region (70º to 90º) to determine the depth, thickness, and concentration of near-surface water/ice.
d. Measurements for water at other locations and depths are not precluded but require further scientific measurements and/or analysis to warrant consideration. This option would specifically include accessing a deep aquifer.
The following investigations are listed in descending priority order.
Investigation 2. Determine the possible toxic effects of martian dust on humans.
Measurements:
a. For at least one site, assay for chemicals with known toxic effect on humans. Of particular importance are oxidizing species such as CrVI. (May require Mars Sample Return (MSR)).
b. Fully characterize soluble ion distributions, reactions that occur upon humidification and released volatiles from a surface sample and sample of regolith from a depth as large as might be affected by human surface operations.
c. Analyze the shapes of martian dust grains sufficient to assess their possible impact on human soft tissue (especially eyes and lungs).
d. Determine if martian regolith elicits a toxic response in an animal species that are surrogates for humans.
Investigation 3. Assess atmospheric electricity conditions that may affect TAO and human occupation.
Measurements:
a. Basic measurements:
i. DC E-fields 0-80 kV/m, dV=1 V, bandwidth 0-10 Hz, rate = 20 Hz
ii. AC E-fields 10 uV/m – 10 V/m, Frequency Coverage 10 Hz-200 MHz, rate = 20 Hz, with time domain sampling capability
iii. Atmospheric Conductivity 10-15 to 10-10 S/m, ds= 10% of local ambient value
iv. Ground Conductivity > 10-13 S/m, ds= 10% of local ambient value
v. Grain charge >10-17 C
vi. Grain radius 1-100 um
b. Combine with surface meteorological package to correlate electric forces and their causative meteorological source > 1 martian year, both in dust devils and large dust storms. Combine requirements for 1Bb with 3a above.
Investigation 4. Determine the processes by which terrestrial microbial life, or its remains, is dispersed and/or destroyed on Mars (including within ISRU-related water deposits), the rates and scale of these processes, and the potential impact on future scientific investigations.
Measurements:
a. Determine the rate of destruction of organic material by the martian surface environment.
b. Determine the mechanisms and rates of martian surface Aeolian processes that disperse organic contaminants.
c. Determine the adhesion characteristics of organic contaminants on landed mission elements, and the conditions and rates under which these contaminants are transferred to the martian environment.
d. Determine the mechanisms to transport surface organic contaminants into the martian subsurface, and in particular, into a martian aquifer.
e. Determine if terrestrial microbial life can survive and reproduce on the martian surface.
Investigation 5. Characterize in detail the ionizing radiation environment at the martian surface, distinguishing contributions from the energetic charged particles that penetrate the atmosphere, secondary neutrons produced in the atmosphere, and secondary charged particles and neutrons produced in the regolith.
Measurements:
a. Measurement of charged particles with directionality. Identify particles by species and energy from protons to iron nuclei in the energy range 20-1000 MeV/nuc.
b. Measurement of neutrons with directionality. Energy range from 1 keV (or lower) to 100 MeV (or higher).
c. Simultaneous with surface measurements, a detector should be placed in orbit to measure energy spectra in Solar Energetic Particle events.
Investigation 6. Determine traction/cohesion in martian regolith (with emphasis on trafficability hazards, such as dust pockets and dunes) throughout planned landing sites; where possible, feed findings into surface asset design requirements.
Measurements:
a. Determine vertical variation in in-situ regolith density within the upper 30 cm for rocky areas, on dust dunes, and in dust pockets to within 0.1 g cm-3.
b. Determine variation in in-situ internal angle of friction of regolith for dust dunes and dust pockets to within 1 degree.
c. Determine regolith cohesion for rocky areas, dust dunes and in dust pockets to within 0.1 kN m-2.
d. Precision imaging to Mars Reconnaissance Orbiter High Resolution Imaging Science Experiment (MRO HiRISE) standards (30 cm/pxl) for selected potential landing sites For basic design of mobility systems, the following measurements are needed (not just at the dust pockets and dunes, but also on the consolidated regolith surfaces where we may do most of the driving): (i) rolling resistance (the torque that regolith applies to a rolling wheel while driving), (ii) traction test (torque required to spin a wheel while the rover is held stationary), and (iii) shape/size of the resulting wheel ruts while driving normally.
Note #11: These three things will probably be measured routinely on all Mars rovers.
Note #12: ISRU excavation could require hauling larger loads (regolith/ice payload) than what we have ever hauled in the past. Therefore they will need these data to properly design wheels and chases (e.g., rover and large structure mobility systems), avoiding energy-wasteful designs or risk of getting bogged down.
Investigation 7. Determine the meteorological properties of dust storms at ground level that affect human occupation and EVA.
Measurements:
a. P, V, T, n, and dust density (opacity) as a function of time at the surface, for at least a Martian year, to obtain an understanding of the possible meteorological hazards inside dust storms. Surface Package measure directly:
i. Same as requirement for 1Bb with added
ii. Dust size 1-100 um
iii. Dust density 2-2000 grains/cc
b. Orbiting weather station: optical and IR measurements to monitor the dust storm frequency, size and occurrence over a year, & measure terrain roughness and thermal inertia. Climate sounder would enable middle atmosphere temperature measurements. In situ density or spacecraft drag sensors could monitor the dust storm atmosphere inflation at high altitudes. Same as requirement 1Bc.
Objective B. Conduct risk and/or cost reduction technology and infrastructure demonstrations in transit to, at, or on the surface of Mars.
Technology validation on Mars flight missions and infrastructure emplacements are needed to reduce the risk and associated uncertainty inherent in new, unproven technologies and to reduce the cost of human Mars exploration. These demonstrations were chosen for Mars robotic flight missions based upon: (a) their high degree of interaction with the Mars environment, (b) their anticipated large leverage on human Mars mission architecture feasibility, and (c) uncertainty of whether Earth, Earth-orbit or lunar testing would supply sufficient data to reduce risk and cost, or increase performance of these systems.
Prioritization Criteria
The following criteria were used, assuming a series of robotic flight missions preceding the first human mission, to establish the priority of importance, when the validation should be done and whether or not the validation must be done on a Mars mission:
1. Priority: The anticipated magnitude of the risk and/or cost reduction of human missions to Mars.
2. Timing: The likely timing of the technology flight mission needed relative to the first human mission:
- Early: to influence architecture decisions (14 to 20 years prior)
- Mid: to influence mission and flight system design decisions (8 to 12 years prior)
- Late: to influence operability decisions (4 to 6 years prior)
3. Venue: Some technology validations can be accomplished less expensively and more comprehensively on other than Mars missions. Required technologies were therefore grouped according to whether they can best be performed (in decreasing order of cost) :
a. At or in transit to/from Mars
b. On the Moon
c. In Earth orbit
d. On Earth
Only those technology and infrastructure items requiring demonstration at Mars, 3a, are listed below as candidates for Mars human precursor missions. Those not requiring development and demonstration on Mars missions, 3b,c and d, are prioritized and described in the associated white paper. Additionally, a set of suggested near-term systems studies has been defined which will, when completed, enable refined definition and prioritization.
The following is a prioritized grouping 1 through 3; there is no sub-prioritization within the groups.
T/I Demonstration 1A. Conduct a series of three aerocapture flight demonstrations:
- 70 deg sphere cone shape (robotic scale) to demonstrate aerocapture at Mars (Early).
- New entry vehicle configuration suitable for human exploration (robotic scale) aerocapture at Mars (Mid).
- New entry vehicle configuration suitable for human exploration (larger scale, end-to-end mission sequence) aerocapture at Mars (Late).
A primary challenge of flight missions to Mars is decelerating the incoming spacecraft. There are four primary options:
- Chemical propulsion
- Aerobraking
- Direct entry
- Aerocapture
Given the expected mass of human missions, the required mass of propellant to use Option #1 is large—this could lead to severe cost and operational problems. Aerobraking (Option #2) is the approach in which multiple orbits are used, extending over months, to gradually lower the apoapsis as was done on the Mars Global Surveyor (MGS) and Odyssey missions. The disadvantage of using this for human missions is that it takes considerable time, which is likely to be incompatible with other constraints on the human mission. Direct entry (Option #3), as used by Pathfinder and Mars Exploration Rover (MER) missions, is at the extreme variation of aeroassist in which entry capsules are decelerated to the point of parachute deployment. However, the “g” forces involved in direct entry are excessive relative to human tolerance. Aerocapture (Option #4) is an intermediate form of aeroassist in which the vehicle is captured into low Mars orbit on the first atmospheric pass. Aerocapture requires accurate navigation, attitude control, and an effective aeroshape design. It is thought that it will take three flight missions to develop this technology to a level of confidence that one would be prepared to use it on a human mission.
T/I Demonstration 1B. Conduct a series of three in-situ resource utilization technology demonstrations:
(1) ISRU Atmospheric Processing (Early)
- Demonstrate acquisition and separation of atmospheric resources for life support & propulsion/fuel cell power systems. Demonstrate production and storage of critical mission consumables (oxygen, fuel, life support gases, etc.).
- Preliminary Experiment Requirements: Mass <25 kg; Power <50 W; Production rate > 20 gm/day O2 or O2/fuel;
- Duration > 30 sols
(2) ISRU Regolith-Water Processing (Early)
- Perform excavation of Mars regolith down to 2 meters, and demonstrate regolith handling and transport capabilities to regolith processing unit. Perform in-situ measurements to support design of robotic and human scale excavation systems. Perform regolith processing to extract and separate water from the regolith in multiple batches to obtain TBD ml of water. Perform in-situ measurements of the water & volatiles released during processing. Perform processing on the water to produce oxygen, hydrogen, and methane.
- Requirement for mobility is dependant on results from ’07 Phoenix and ’09 Mars Science Laboratory (MSL) missions
- Preliminary Experiment Requirements: Mass <30 kg; Power <50 W; Production rate > 10 cm3/day of water;
- Duration > 15 sols
(3) ISRU Human-Scale Application Dress Rehearsal (Late)
- The primary purpose of this demonstration is to “buy down” risk by operating a complete end-to-end ISRU system (1/20 scale, duration 80 sols) culminating in a significant application such as producing propellants for a Mars Hopper, and the subsequent operation of the Hopper. An additional goal is to make a first attempt to gather and utilize regolith for construction purposes, e.g., radiation shielding, at a small scale. This will be closely coupled to excavation, material transport, and beneficiation techniques derived from Regolith-Water Processing demonstration.
- Perform in conjunction with other human mission subsystems if possible, e.g.., RTG power system, fuel cell power system, oxygen/methane propulsion, landing hazard avoidance.
- Preliminary Requirements: 0.25 to 0.05 kg of O2-fuel/hr, Power 200 to 400 W, Duration 80 sols min.
It is not yet known when, if at all, ISRU will be a part of human missions to Mars. However, if ISRU is to be fully assessed, the demonstrations are considered to be required, e.g., they are necessary to reach a decision to proceed or not with ISRU, and constitute yes/no gates. These demonstrations would need to be carried out in parallel with Goal IVA Investigation #1D, which relates to discovery and characterization of the water deposits.
Currently there are no criteria for where human exploration will occur on Mars. If ISRU is a potentially enabling factor in affordability or safety, then resource location and feasibility will be a driver in site selection. Alternatively, if a negative decision regarding ISRU were to be made on the basis of relative cost or risk alternatives, then these demonstrations become moot.
In addition to the exploration-related questions in Goal IVA-1D, the practicalities of implementing ISRU are serially dependent upon two fundamental factors: 1). Is the potential resource “economically” accessible, meaning can enough be procured at acceptable cost, to more than offset the cost of bringing it from Earth? 2). Can sufficient supplies of the end-product be produced, stored and transferred with low risk? The most obvious uses of in situ resources are those that focus on obtaining resources that, if they have to be launched from Earth, are large mass, thus cost, drivers. Typically water, oxygen and fuels are leading candidates for ISRU. Water has immediate use as such for crew life support and hygiene. It also serves as a source for hydrogen and oxygen. The atmosphere of Mars contains carbon dioxide, traces of water vapor, and inert gases (useful for diluting atmospheric oxygen). The carbon dioxide can be a source of oxygen and can be reacted with hydrogen to make fuel.
T/I Demonstration 1C. Demonstrate an end-to-end system for soft, pinpoint Mars landing with 10m to 100m accuracy using systems characteristics that are representative of Mars human exploration systems. (Mid)
Pinpoint landing systems for human exploration vehicles are required for two reasons. First, safety and risk mitigation. It is likely that pre-positioned supplies and emergency abort systems will be located on Mars prior to arrival of humans. Landing near such assets increases the likelihood of successfully accessing such in an emergency and enhances mission efficiency in non-emergency situations. Second, it is likely that a site selected for human exploration will be selected because there is a specific science objective to be accomplished. Such could be sufficiently localized that landing in proximity increases the probability of successful science accomplishment.
T/I Demonstration 2A. Demonstrate continuous and redundant in-situ communications/navigation infrastructure (Early). Deploy in full-up Precursor Test Mission (Late).
Mission safety and effectiveness will require the development of high band-width continuous and redundant communications. This support for human mission can be met, e.g., by a pair of longitudinally-offset areostationary relay satellites
T/I Demonstration 2B. Investigate long-term material degradation over times comparable to human mission needs. (Mid)
Our current state of knowledge of the Mars environment is inadequate to confidently design essential space systems to be used on the martian surface. These include EVA suits, habitats and ancillary systems, including mobility.
It is essential to verify the capability of materials under consideration for Mars surface operations to tolerate long term exposure (years) to Mars environmental phenomena using coupon, component, subsystem and system level tests on Mars. Phenomena include: radiation; temperature extremes and cycles; wind; atmosphere chemical and electromagnetic properties; regolith and dust chemical, mechanical, and electromagnetic properties; and Mars biology (if any). An LDEF (Long Duration Exposure Facility) should be considered (implied return of test samples).
T/I Demonstration 3. Develop and demonstrate accurate, robust and autonomous Mars approach navigation. (Mid)
This is a mission safety item. It would provide a backup/replacement to Deep Space Network (DSN)-based terminal navigation for a mission time-critical event (e.g., Mars Orbit Insertion (MOI) or aerocapture).